"It is all about communication" – a phrase that is fully applicable also in the world of quantum computing. Here, the information carriers are qubits, not people; and they can only exchange information when brought into close proximity. How exactly do qubits exchange information, and how can this exchange scale within the number of qubits?
Picture a future where Quantum Processing Units (QPUs) powered by spin qubits reach a milestone of a million qubits. Even in such a QPU, all quantum operations, regardless of the number of qubits involved, boil down to gate operations between pairs of qubits. When qubits are physically close, for instance, in a double quantum dot device, they can directly interact and influence each other's quantum information, eliminating the need for long-distance transfer. As quantum systems evolve beyond just a few qubits to systems with hundreds or thousands of qubits, we will start to see the initial benefits of quantum computing in the NISQ era. However, scaling up to a many-qubit QPU, capable of running complex quantum algorithms, presents an entirely new level of complexity. To visualize this, think of a postman delivering crucial messages (quantum information) between houses. The postman arrives at a house (representing a qubit), briefly interacts to deliver the message (a gate operation), and then both the postman and the resident may take separate actions. For instance, the resident might discover a parking ticket in the delivered mail and need to handle it, while the postman moves on to the next house to continue his deliveries. This cycle repeats across many stops. The postman never stays at any one house - he simply acts as a carrier, enabling communication and then moving on.
Similarly, spin qubits can be transported to interact with others to exchange quantum information, and then continue to further interactions for further information exchange. The question arises: How can this transport be achieved without losing the quantum information encoded in each spin?
Spin shuttling as quantum’s messenger
Spin shuttling is the method to achieve this qubit transport. This technique stands out for its remarkable ability to preserve quantum information. Essentially, spin shuttling involves moving a charge using gate potentials. By enabling qubit interactions, shuttling facilitates the transfer of quantum information both between and within arrays of qubits, as detailed in research such as in Vandersypen et al. 2017. Recent advancements have even demonstrated maintained qubit coherence during high-fidelity single-spin shuttling, as seen in De Smet et al. 2024 and Foster et al. 2024.
When it comes to shuttling methods, two main approaches exist: Bucket-brigade mode and Conveyor mode.
Bucket-brigade mode acts as a reliable base approach, especially in scenarios where precise, localized control is critical. In this mode, qubits are moved between nodes in a sequential, step-by-step manner. Each node temporarily holds the qubit before passing it to the next. This method is well-suited for architectures where qubits only need to travel short distances or where operations are localized.
However, this sequential handoff process can introduce delays, and maintaining qubit coherence across multiple transfer steps becomes increasingly challenging. As the qubit count grows, the inherent delays and coherence challenges in Bucket-Brigade mode can become bottlenecks, especially for large-scale applications requiring speed and coherence over extended distances.
Conveyor mode provides a key alternative: moves qubits along a defined pathway in a continuous motion, with the flexibility to pause at any point if necessary. This method is often preferred in systems where qubits need to travel varying distances or where continuous transport can improve operational efficiency. For systems with a large number of qubits, the Conveyor mode can significantly boost the efficiency of quantum communication by reducing the time it takes to shuttle qubits over long distances.
When it comes to protecting quantum information over longer distances and time scales, Conveyor mode can offer some advantages for scaling compared to Bucket-Brigade mode. Bucket-Brigade mode typically transfers spins step by step through multiple tunnel junctions, which can introduce cumulative errors, dephasing, and increased susceptibility to charge noise. Conveyor mode, in contrast, moves the spin within a single, smoothly shifting potential well, minimizing transitions, reducing decoherence, and ensuring more stable quantum information preservation over extended distances and durations.
Qblox brings spin qubits together
Bucket-brigade mode or Conveyor mode; two different ways to shuttle the spin qubits? Qblox can make this happen. The Qblox Cluster setup can be tailored to meet specific device requirements, covering a frequency range from DC to 18.5 GHz. This setup includes the modules Qubit Readout and Control (QRC), Qubit Control Module (QCM), Qubit Control Module RF (QCM RF II), and Qubit Readout Module (QRM), providing flexible control outputs and readout inputs for precise operation across various quantum computing systems. Additionally, the latest Qblox DC Cluster mainframe features the Quantum Source and Measurement (QSM) module, which integrates sourcing and measurement capabilities into a single instrument. Whether for microwave or baseband pulses for qubit drives, the configuration can be customized to achieve optimal performance.
How the shuttling is performed:
- In Bucket-brigade mode, connected by gates, represented by a chain of quantum dots. Each dot is defined by a static DC voltage, ensuring long-term signal stability. Before starting, every quantum dot must be calibrated individually, ensuring smooth transitions from one dot to the next. The energy levels of adjacent dots are adjusted in a synchronized manner to allow for an adiabatic transition of the charge from one dot to the other.
- Conveyor mode: The journey begins at a single entry point—a quantum dot connected to a reservoir. From there, a moving potential is created using four generated sinusoidal waves, allowing the qubit to glide continuously along the track. This can be done by the Qubit Control Module (QCM). The parameters of the sinusoidal waves - phase, frequency, and amplitude - are dynamically controlled by Qblox’s parameterized operations, allowing for adjustments during the experiment to maintain qubit coherence.
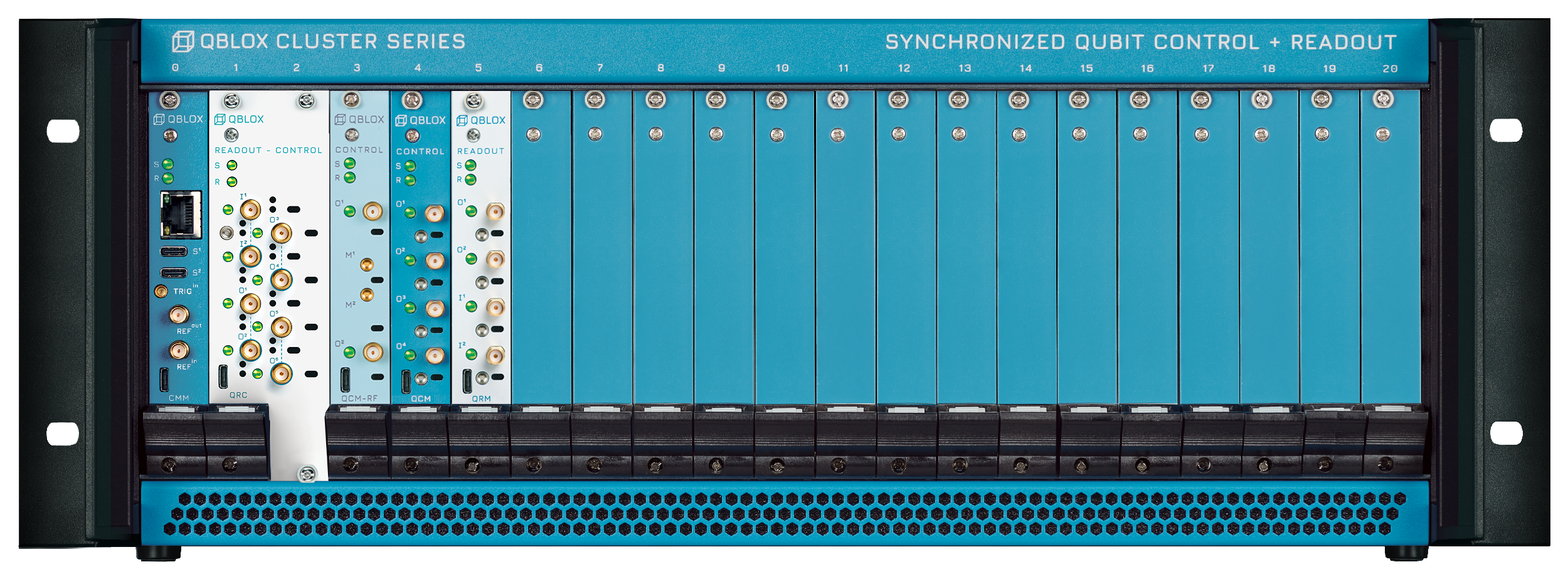
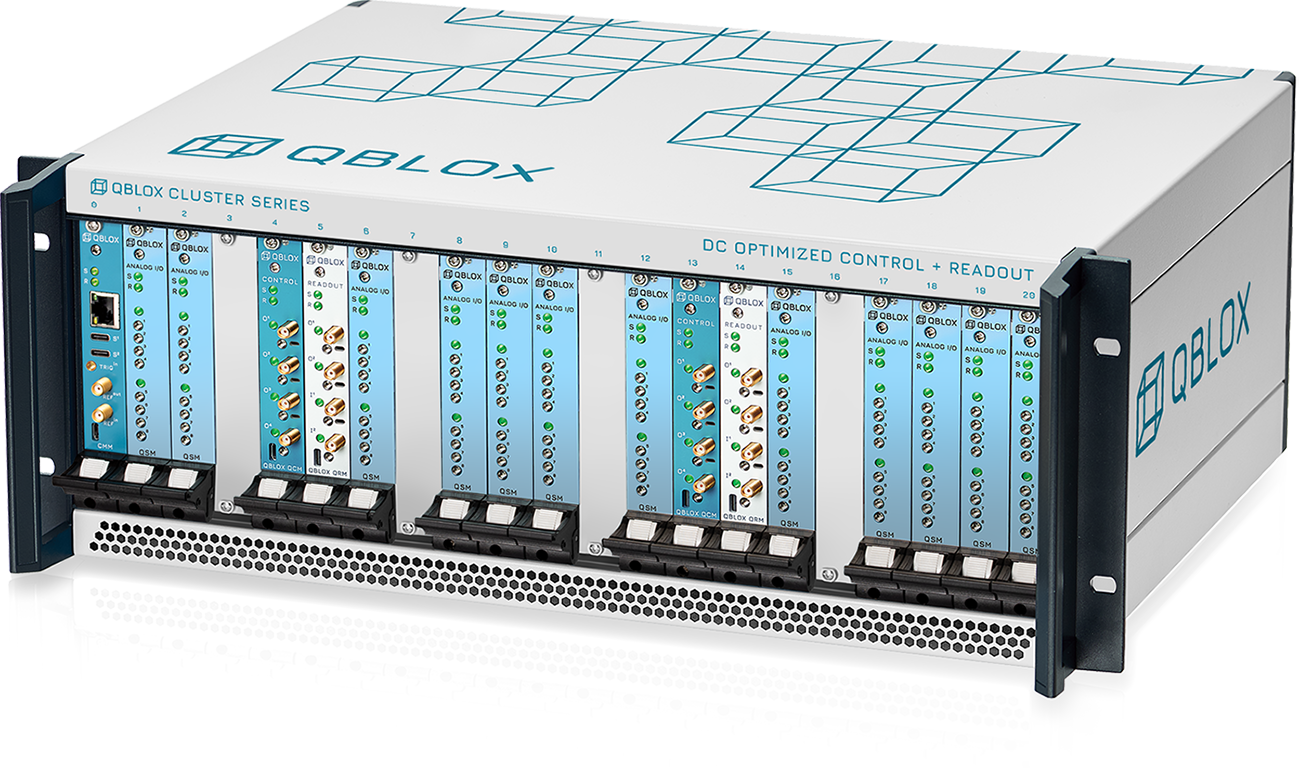
Spin-qubit shuttling with Conveyor mode
Let's walk through a typical two-qubit gate experiment with distant qubits to understand how spin qubits are transported. The goal is to initialize a spin, shuttle it to interact with another initialized spin, perform a two-qubit gate operation to exchange information, and then shuttle it back for readout to analyze the final state. In this process, an electron spin is accelerated by a moving electrostatic potential created by sinusoidal waves. This spin is moved along a track, and paused to perform a two-qubit gate operation with another spin. The two spin qubits can now ‘talk’ and exchange encoded spin data. The shuttling direction is then reversed, returning the spin to its starting point for readout. This round-trip allows for the assessment of the two-qubit gate's impact and the effects of shuttling.
The amplitude, frequency, phase, and offset of the sine waves are dynamically adjusted by Qblox’s Qubit Control Module (QCM). Imagine needing four sinusoidal waves for each conveyor, and then multiple conveyors operating simultaneously. This will require separate output channels that are well synchronized with each other to nanosecond precision. To ensure these operations are fully deterministic, Qblox's Q1 sequencer provides the necessary control, while Qblox's SYNQ protocol ensures synchronization between multiple channels, enabling the high-precision control needed for successful spin-qubit shuttling.
Qblox taking spin shuttling to the next level
Success in spin-qubit shuttling relies on precise synchronization and modularity. Transmitting quantum information over long distances requires coordination to prevent loss of coherence. Qblox's technology is valuable given the sequential operation mentioned above for the 2-qubit gate experiment, which involves multiple operations. It showcases the power of gapless sequencing and the 1-nanosecond time grid, enabling precise, high-speed operations crucial for maintaining qubit coherence. These features enable high-speed operations and precise manipulations necessary to keep qubits in their desired states.
Qblox's control stack electronics are specifically designed to address the challenges of synchronizing large-scale systems. The ability to synchronize multiple control and readout channels ensures accurate manipulation of each qubit, even as the system scales up. The simplicity of the Qblox design maintains high fidelity and accuracy, regardless of system size, empowering researchers to push the boundaries of quantum computing.
.gif)
Are we ready to scale up?
Scaling up qubits is an exciting prospect, but it also raises critical questions about how we’ll maintain coherence, minimize errors, and ensure efficient communication across vast networks of qubits. Spin qubits will need to support more complex, high-speed operations without compromising performance.
Current demonstrations are limited to one-dimensional shuttling, while the true potential of spin-qubit networks lies in expanding to 2D. This expansion will require a system capable of directing spin qubits along more intricate paths, allowing them to “turn left or right” and interact with each other in new ways. However, this approach will require careful engineering and innovative solutions to ensure that the quantum information is preserved during these more dynamic transfers. At the same time, progress is being made to develop the materials needed for successful shuttling. Highly purified, defect-free surfaces are critical for maintaining qubit coherence during long-distance transfers. Research in materials such as SiMOS and germanium-based systems is essential in overcoming these hurdles.
With continuous improvements in qubit shuttling techniques and the ongoing development of better materials, the scaling of spin-qubit systems is no longer a distant dream. It is becoming a reality, thanks in no small part to the tools and technologies being developed today. Qblox, with its precise control and modular architecture, is helping researchers to build quantum systems that will ultimately support large-scale spin-based QPUs, heralding a new epoch in quantum computing.
Qblox empowering tomorrow’s quantum visionaries
The Qblox’s 1 ns time grid adds another layer of precision, particularly important when tuning voltage levels at plunger gates. No other system currently offers this level of time-grid resolution, making Qblox a unique enabler for the fast, high-fidelity operations required in spin-qubit shuttling. Qblox’s modular design, coupled with the SYNQ protocol, provides full synchronization across multiple channels. Whether the system operates in conveyor mode or bucket-brigade mode, the architecture remains consistent, supporting smooth transitions between experimental setups. For us, the evolution of spin-qubit shuttling isn’t just about pushing the boundaries of control. It’s about empowering the next generation of quantum scientists and engineers to build the infrastructure that will power future quantum processors. Through precise timing, advanced synchronization, and a modular approach, Qblox is at the forefront of supporting these visionary scalable experiments.
In the coming years, we will likely see these systems take shape, moving from conceptual designs to fully functional processors that push the boundaries of computation. It’s an exciting time to be involved in quantum research, and with Qblox’s support, tomorrow’s quantum visionaries will be well-equipped to tackle the challenges of scaling up.
Qblox Spin Success Story
High-Fidelity Single-Spin Shuttling in Silicon
Researchers at QuTech, Delft, have achieved high-fidelity shuttling of a single electron spin in a silicon quantum dot array, a significant step toward large-scale quantum computing. Using Qblox control electronics, including the QCM, QRM, and DC sourcing module, the team transported an electron spin over 10 micrometers in under 200 nanoseconds with an average fidelity of 99%. This demonstrates the potential of silicon-based spin qubits and the effectiveness of Qblox in enabling precise, high-speed quantum operations.
Read more about this one and other Qblox success stories here.